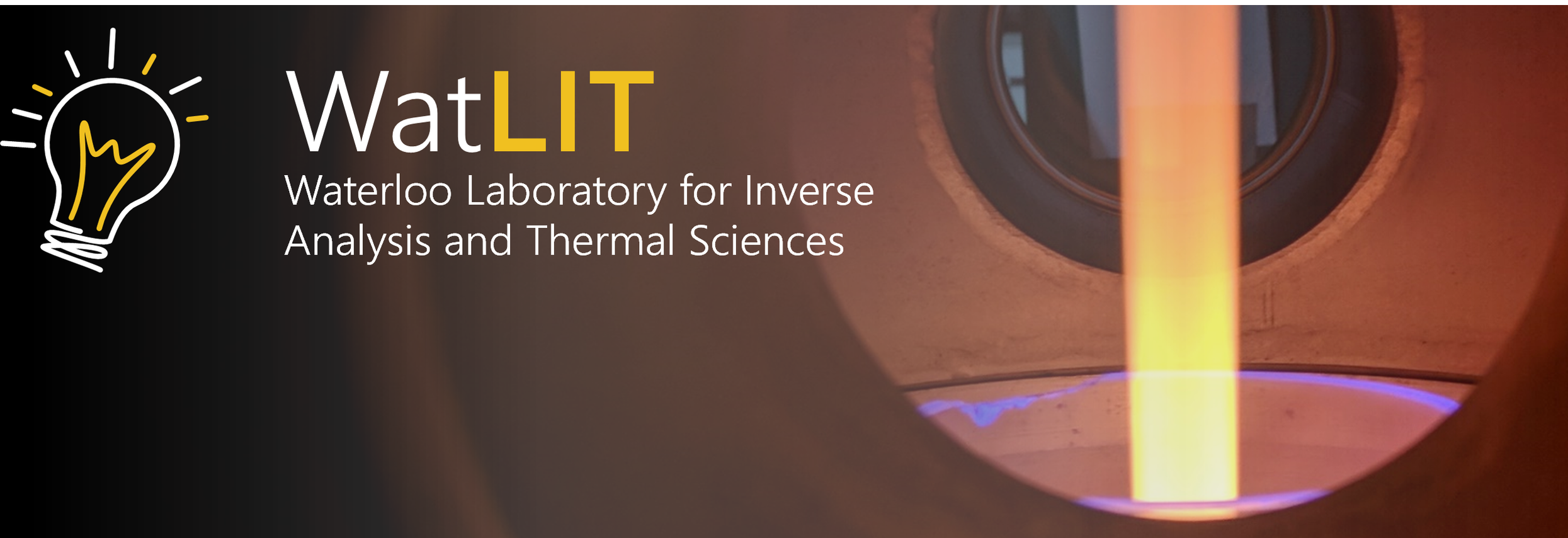
Why is this important?
Nanoparticles feature prominently at the frontiers of science and engineering, due to their unique, size-dependent, chemical and electromagnetic properties. Gas phase synthesis routes are the most promising for large scale production, but nanoparticle size must be carefully controlled to ensure product functionality.
On the other hand, there is growing awareness that many nanoparticles are toxic and threaten the environment. Black carbon, for example, is the second largest climate forcing agent after CO2, and has a particularly pronounced effect on climate change in the Arctic.
Because the positive and negative impacts of nanoparticles depend strongly on their size and shape, scientists and engineers need diagnostics that can provide spatially- and temporally-resolved measurements of nanoparticle concentrations, size, and morphology. Our research group uses time-resolved laser-induced incandescence (TiRe-LII) to determine the size of gas-borne nanospheres, and multiangle light scattering (MALS) to determine the size and shape of fractal-like nanoaggregates, like soot.
Time-resolved laser-induced incandescence (TiRe-LII)
TiRe-LII uses a laser pulse to heat aerosolized nanoparticles within a small probe volume to incandescent temperatures. A common setup involves a laser beam oriented perpendicular to a detector assembly, which most often consists of photomultiplier tubes (PMTs) equipped with bandpass filters aligned to wavelengths in the visible and near-infrared spectrum. The intersection between the laser beam and detection optics forms a probe volume.
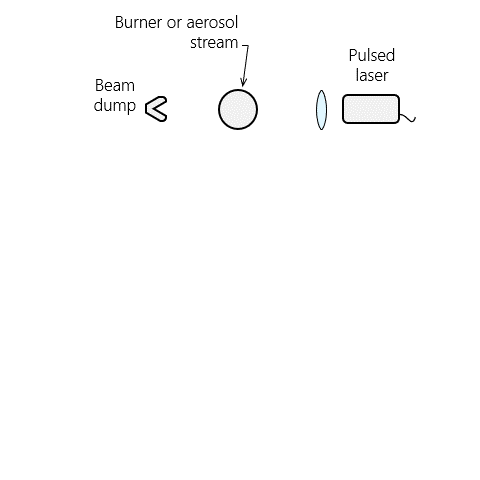
The spectral incandescence of the nanoparticle ensemble is measured as the nanoparticles return to the ambient gas temperature. Since larger nanoparticles cool more slowly than small particles, the particle size distribution can be determined from the observed spectral incandescence decay rate.
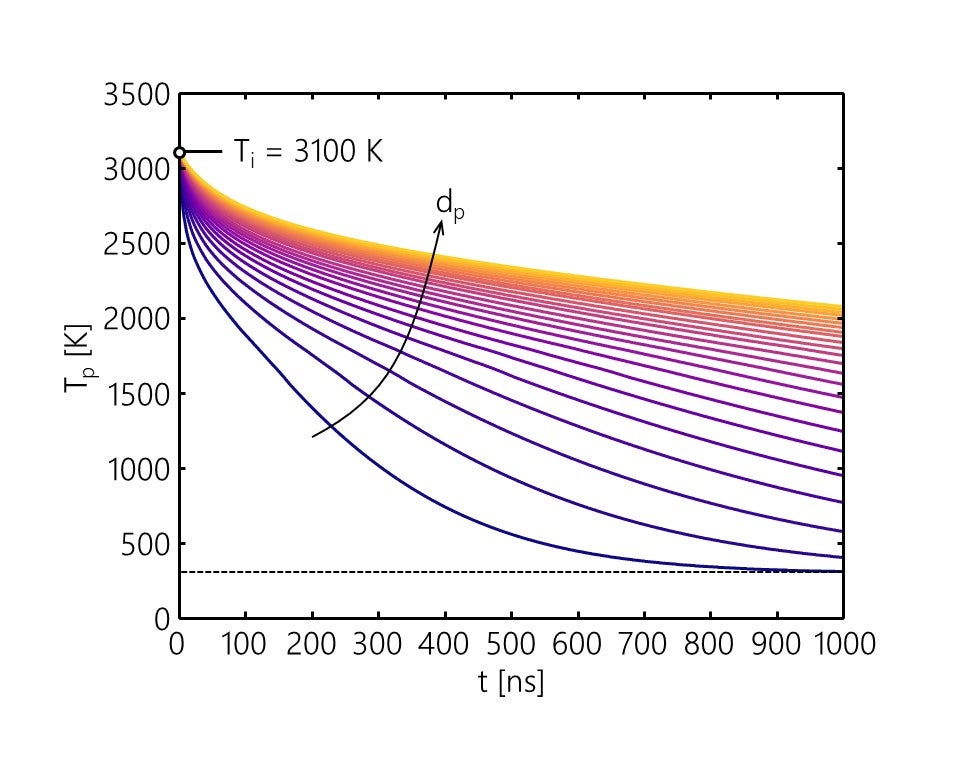
The TiRe-LII model consists of two sub-models: a spectroscopic sub-model, which relates the measured incandescence to the nanoparticle temperatures; and a heat transfer model, which predicts the nanoparticle cooling rate.
TiRe-LII: Spectroscopic sub-model
The spectral incandescence emitted by an ensemble of laser-heated nanoparticles is given by

where dp is the nanoparticle diameter, Qabs,λ(dp) is the spectral absorption cross-section, Iλ,b[Tp(dp)] is the blackbody spectral intensity at a temperature Tp, p(dp) is a probability density function for the nanoparticle diameters, and coefficient C accounts for the particle number density and collector solid angles..
TiRe-LII: Heat transfer sub-model
Nanoparticle temperatures are found from an energy balance that relates the sensible energy of the nanoparticle to laser heating and conductive and evaporative cooling
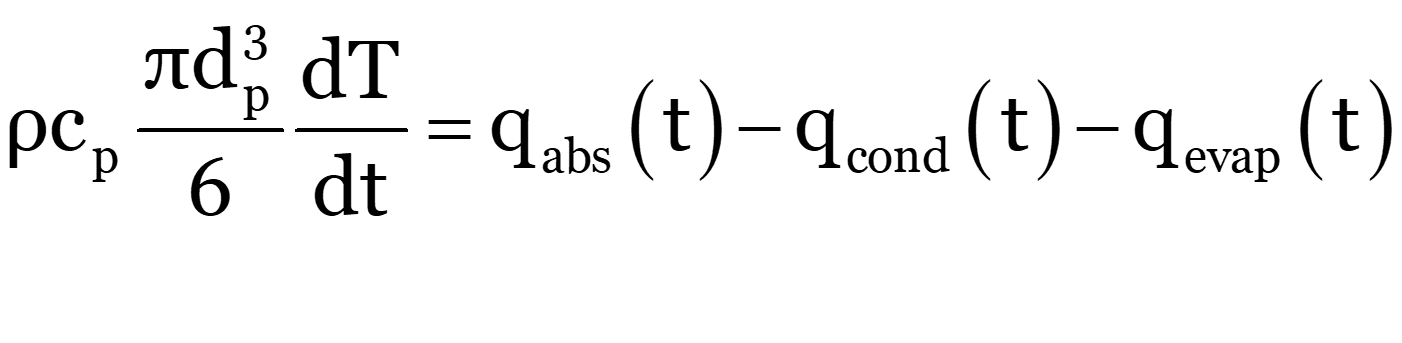
Other cooling terms, including radiative and thermionic heat transfer, are usually at least one order of magnitude lower than conduction and evaporation, and can be neglected from the model.
Conductive and evaporative cooling usually occurs in the free molecular regime (FMR), in which molecules travel between the nanoparticle and the equilibrium state of the gas without colliding with other gas molecules. FMR conduction heat transfer is given by
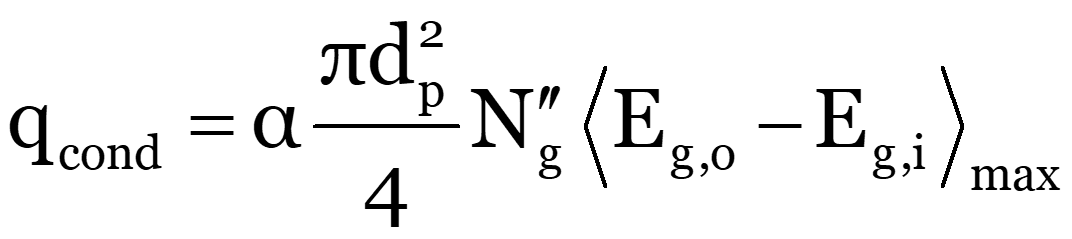
where Ng² is the incident gas molecular number flux, <Eg,o-Eg,i>max is the maximum average energy transferred when a gas molecule scatters from the nanoparticle allowed by the 2nd Law of Thermodynamics, and α is the thermal accommodation coefficient (TAC), which defines the energy transfer efficiency between the gas molecule and the nanoparticle surface.
Evaporative heat transfer is given by
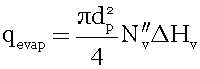
where Nv² is the number flux of evaporated molecules (calculated from the Clausius-Clapeyron equation) and ΔHv is the latent heat of vaporization, which is the energy required to liberate one molecule from the nanoparticle.
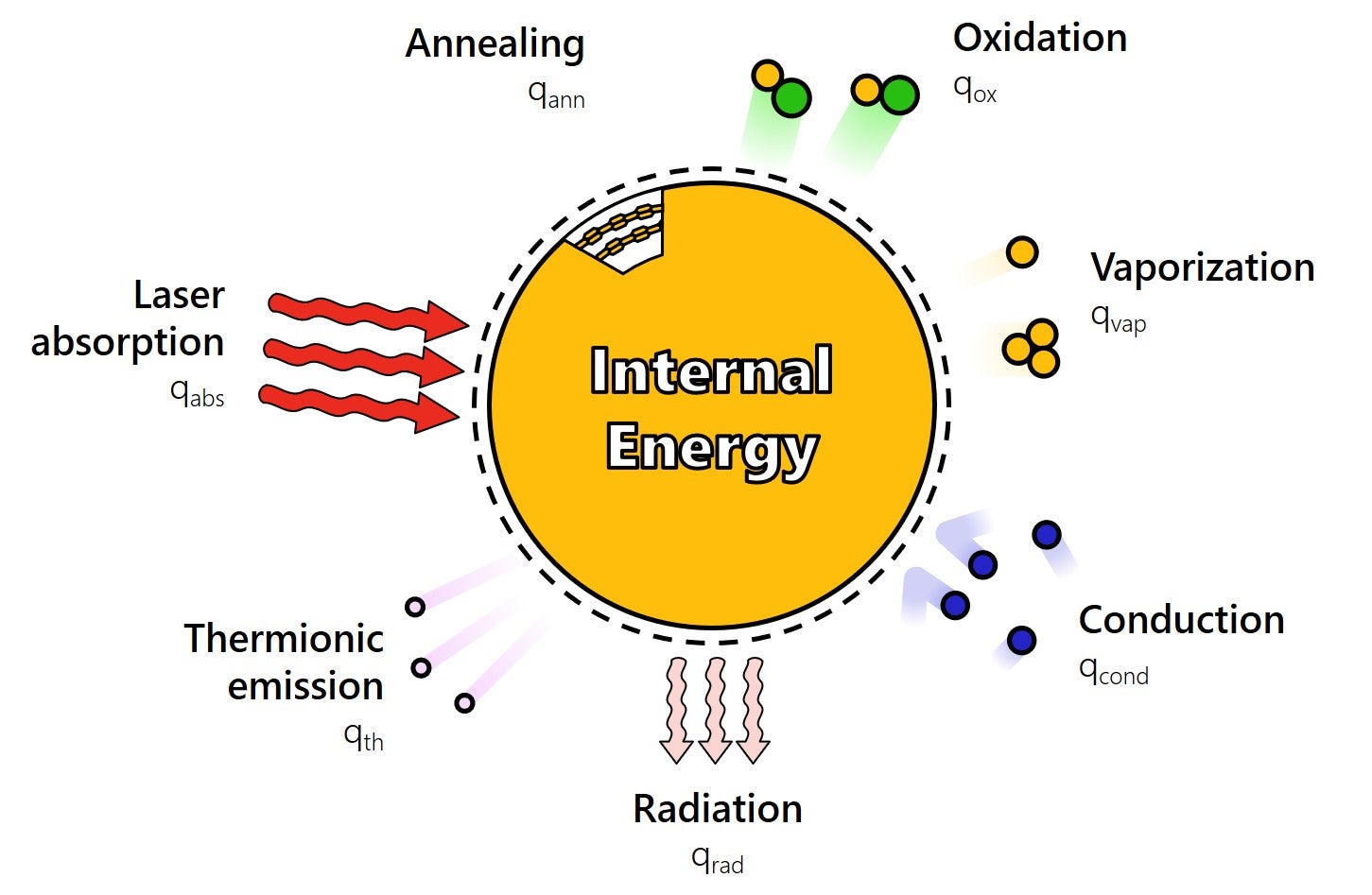
TiRe-LII: Applications
TiRe-LII is most commonly applied to soot, and much of the literature devoted to the in situ investigation of soot in flames, and black carbon in the atmosphere.
There is growing interest in measuring the properties of synthetic nanoparticles, including those made of iron, nickel, molybdenum, silver, gold, silicon, and germanium. While TiRe-LII is mainly used to infer particle size, the diagnostic is increasingly used as a tool for scientific inquiry, e.g. to characterize the TAC, ΔHv, and the electromagnetic properties of liquid metals and molten semiconductors at high temperatures. Recent work has focused on characterizing few layer graphene (FLG), used to enhance the performance of batteries and supercapacitors.
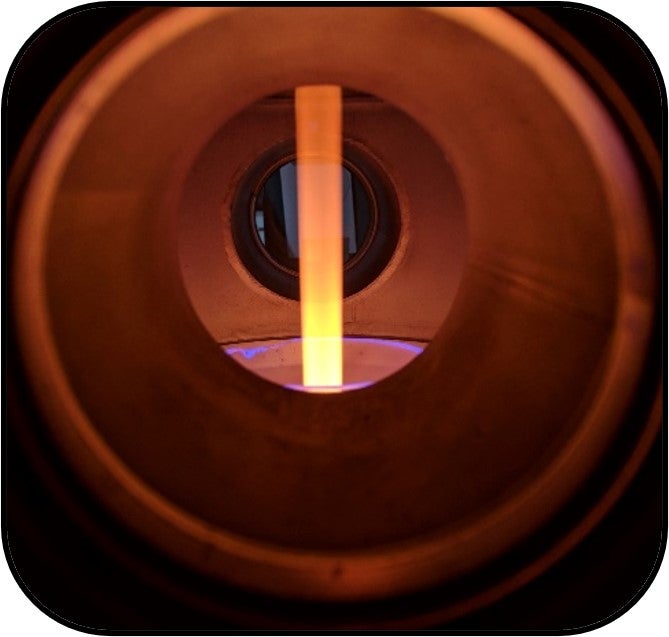
Molten germanium nanoparticles in a microwave plasma reactor at the University of Duisburg-Essen
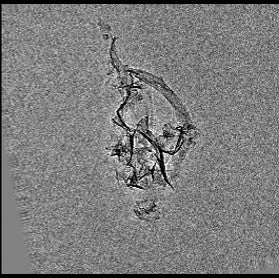
Tomographic TEM images of few layer graphene (FLG) nanoparticles
Multiangle light scattering (MALS)
In many application, aerosolized nanospheres assemble into fractal-like nanoaggregates. In this scenario the size of the aggregate is given by the gyration radius, Rg, which is related to the number of primary particles in the aggregate, Np by the fractal relationship
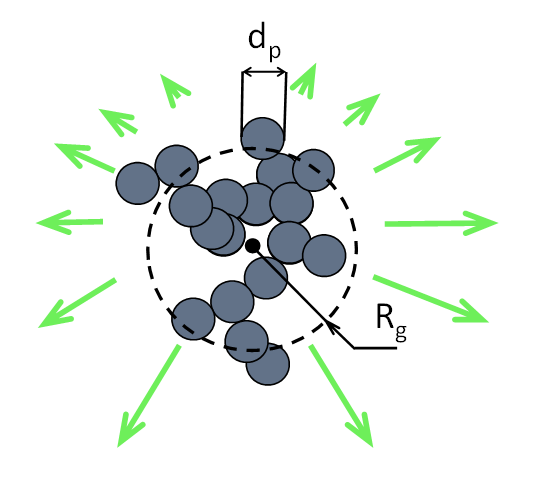
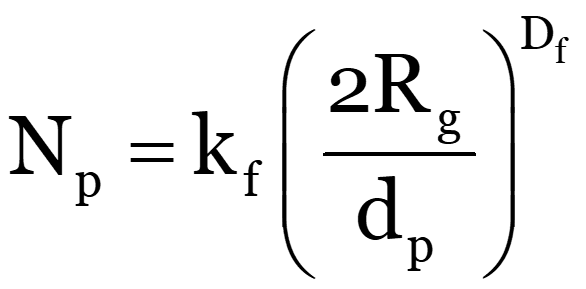
In MALS a laser is shone through the aerosol and the scattered light is measured at various angles. The angular distribution of scattered light is related to the aggregate size distribution

where p(Rg) is the aggregate size distribution and k(Rg, dp, kf, Df) is the light scattering kernel, which is derived from electromagnetic theory. Inferring p(Rg) is mathematically ill-posed so small amounts of measurement noise and model error are amplified into large errors in the recovered aggregate size distribution.
WatLIT has developed new inversion algorithms to minimize this error and improve the reliability of this diagnostic, including light scattering kernels derived using an artificial neural network.
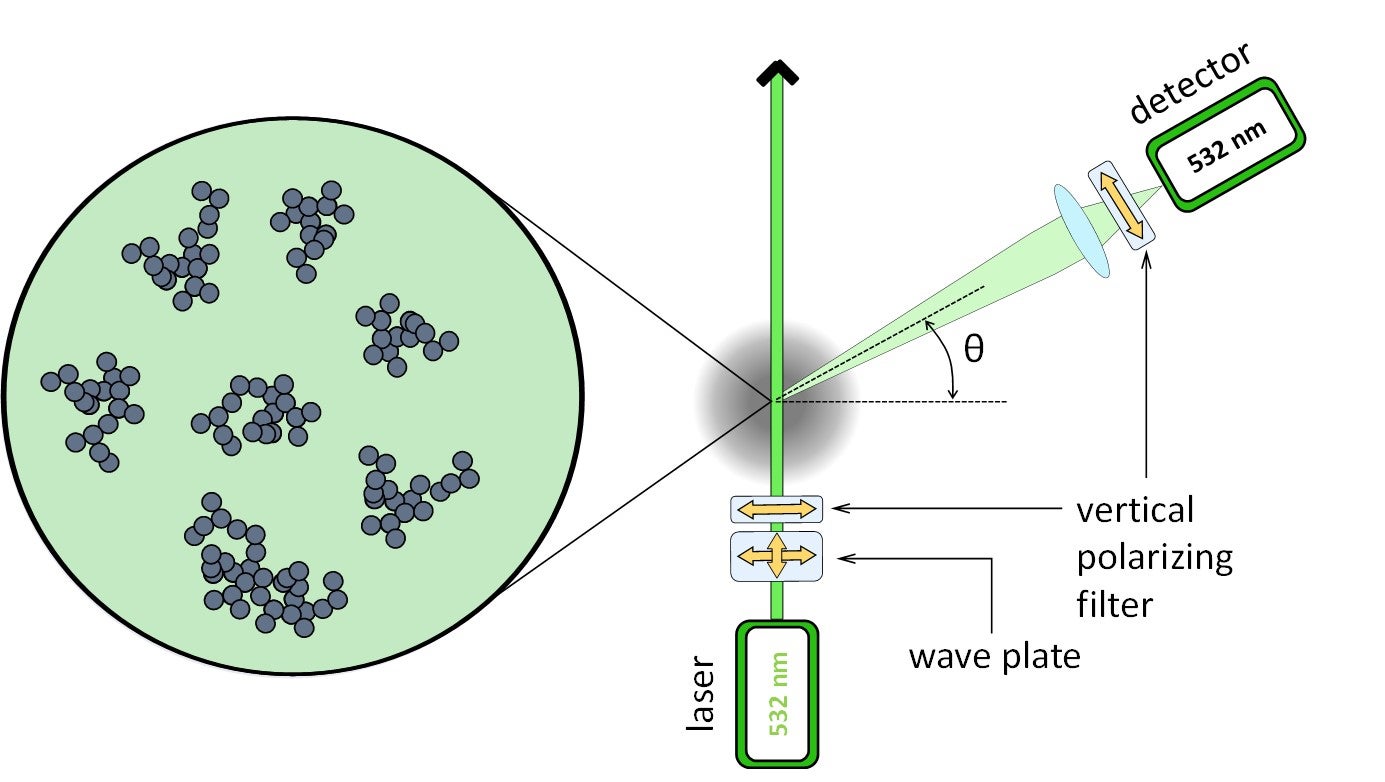
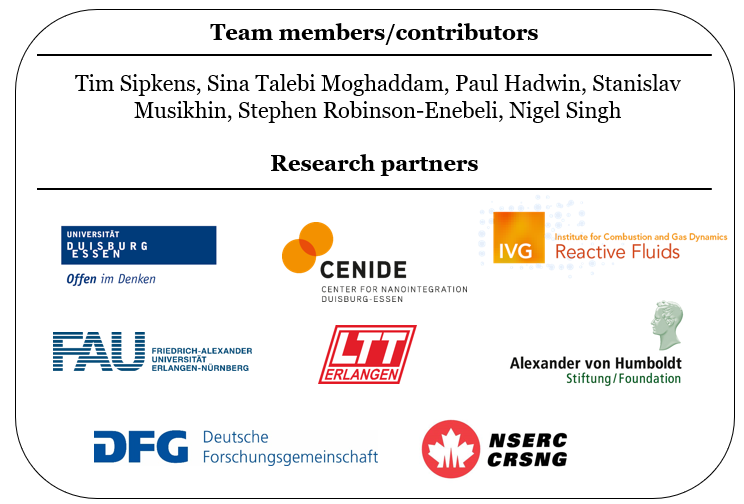