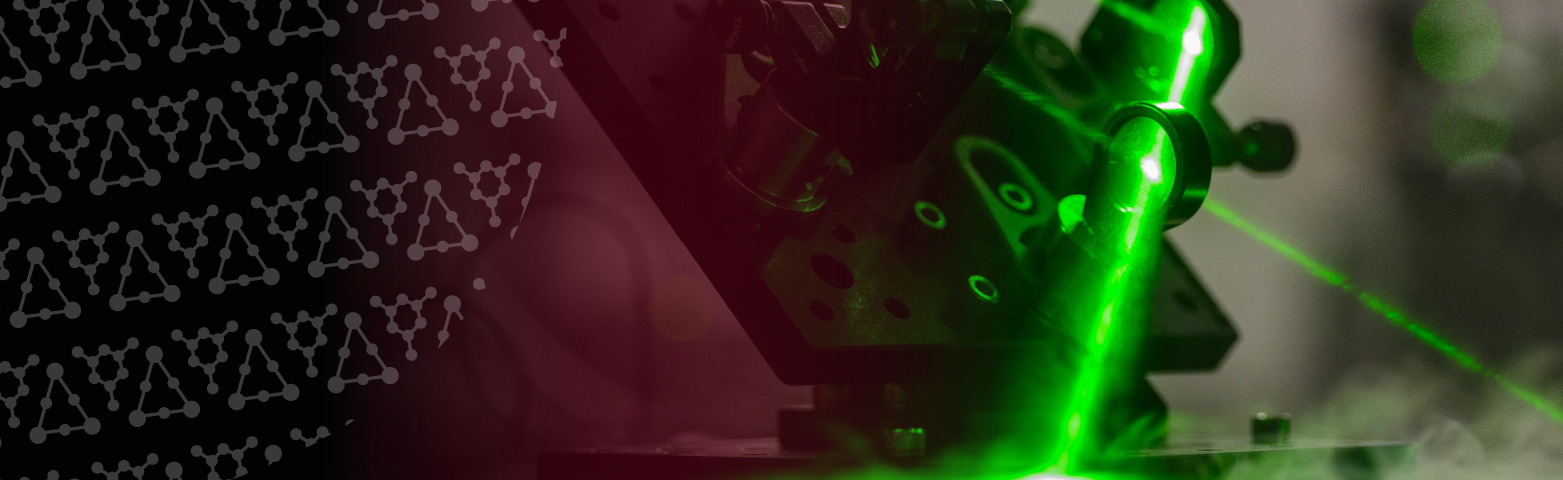
Our Quantum Reality
Quantum mechanics offers us a way to describe the atomic world to a surprising degree of precision. Its predictions often defy our intuition of the world.
All of the remarkable and surprising quantum phenomena come from the fact that probability is at the heart of quantum mechanics. We can never know what will happen for sure. We can only know how likely it is that something might happen.
The Basics of Quantum Mechanics
THE TWO GOLDEN RULES OF QUANTUM MECHANICS
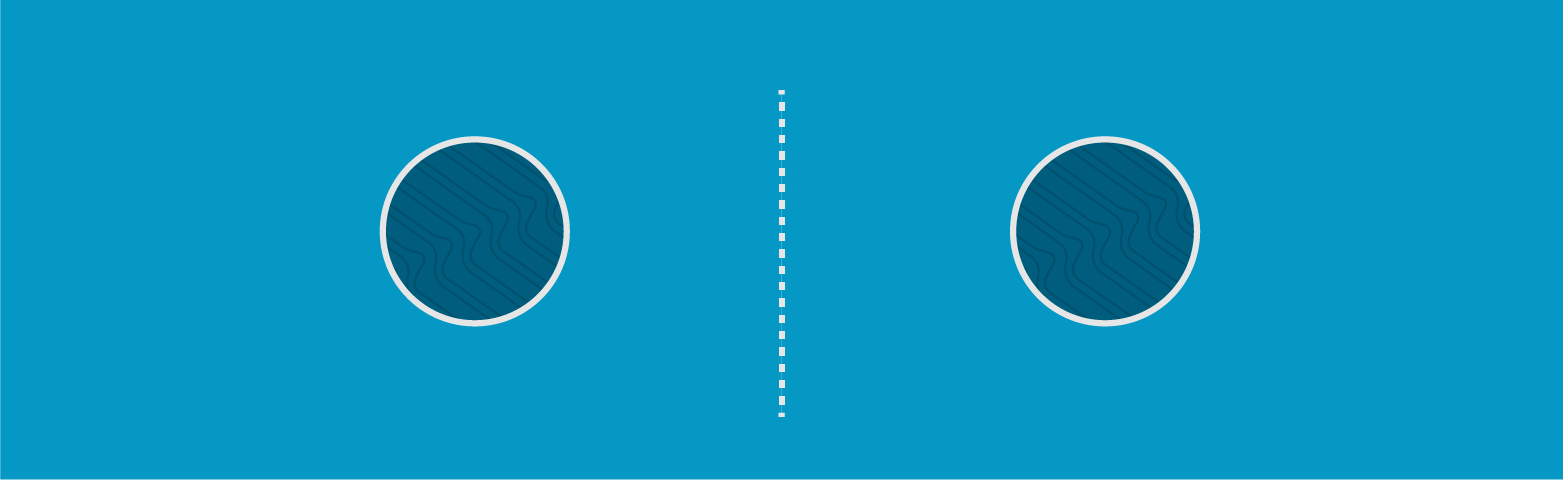
Rule 1: A particle can be in quantum superposition where it behaves as though it is both here and there.
Rule 2: When measured, the particle will be found either here or there.
Many phenomena predicted by quantum mechanics can be understood using these two simple rules. These rules apply to position as well as many other quantum variables like momentum, spin and polarization. They can also be extended to more than two possibilities.
WAVE-PARTICLE DUALITY
In the early 1900s, scientists thought matter was made of particles and light acted like a wave. We now know this is not the case. Light can only be emitted in units called photons, meaning that we can count light like we would count marbles. Large molecules can produce constructive and destructive interference patterns just like water waves. Wave–particle duality is the idea that matter and light can behave both like waves and particles.
The wave-particle nature of quantum objects is described through a wavefunction. Instead of being a wave of energy like a sound wave or a water wave, the wavefunction is a wave of probability. Understanding quantum wavefunctions allows us to understand the random nature of the microscopic world. The key point is a wavefunction considers all possibilities of what might happen at the same time and their relative probabilities. The wavefunction is a central concept of quantum mechanics and leads to many strange consequences.
QUANTUM SUPERPOSITION
Superposition is a property of waves that says if we add waves together we get another, new wave.
You are already familiar with superposition! Think of the strings on a guitar. The A, B and E strings on a guitar each create a sound wave. When we add these together we get a new sound wave – a chord.
Because quantum particles have a wave-like nature, they can be in a quantum superposition of different states. For example, if a particle is in a superposition of two locations, it acts as if it is in both locations at the same time – that is, something can be “here” and “there,” or “up” and “down” at the same time.
INTERFERENCE
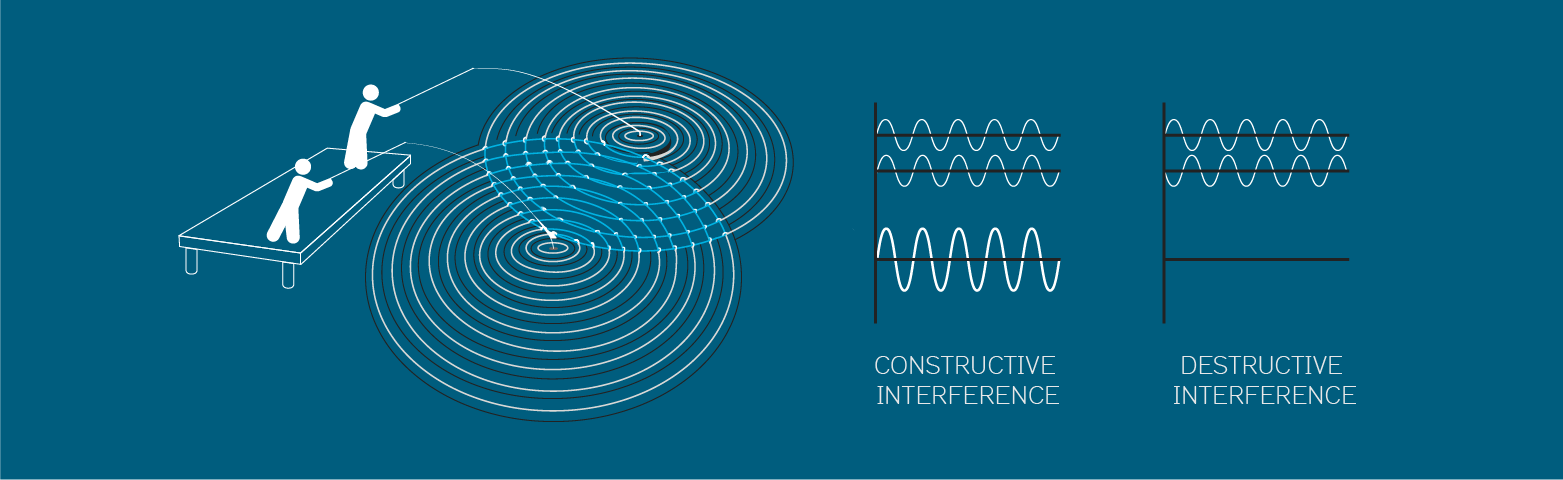
Interference happens when two or more waves meet. Their highs and lows can build on one another, called constructive interference, or cancel each other out, called destructive interference.
In the quantum world, because particles behave like waves, quantum interference comes into play. Constructive interference increases the probability of certain things, such as finding the particle in a certain location, while destructive interference reduces that probability.
Activity: Measuring the Width of a Hair
A human hair is too thin to measure with a ruler. Can we measure it using light interference? Try this activity at home to find out.
QUANTUM MEASUREMENT AND THE UNCERTAINTY PRINCIPLE
In the classical world, we can measure the same object in multiple ways. If I have an apple, I can measure its colour, size and weight, all with the same apple. Measuring the colour of the apple shouldn’t suddenly change its weight.
In the quantum world, we can’t always measure the same object in multiple ways. One famous example is that we can only precisely measure the position of an electron if we ignore its momentum. This phenomenon is known as the uncertainty principle, which describes how measuring one property of a quantum particle unavoidably disturbs another property.
Quantum uncertainty is fundamental to the study of quantum information. If we have a two-sided coin in the classical world, there’s only one way to measure it: is it heads or tails? A quantum coin, on the other hand, can be measured in infinite incompatible ways by measuring whether it's heads, tails, or a superposition of both.
QUANTIZATION
Quantization refers to the fact that only certain discrete values of a property are allowed. For example, the discrete height you reach on a flight of stairs is different than the continuous height you can reach on a ramp.
In music, a guitar string can only vibrate at specific frequencies called harmonics, as all other possible wave frequencies destructively interfere. In quantum mechanics, we see similar behaviour for light, atoms, and more.
Quantization of quantum properties is a consequence of the wave-like nature of particles. The properties of confined quantum particles, such as their energy, are limited to discrete or “quantized” values that could otherwise be continuous. The sharp colours of a neon lamp are an example of quantization that you can see.
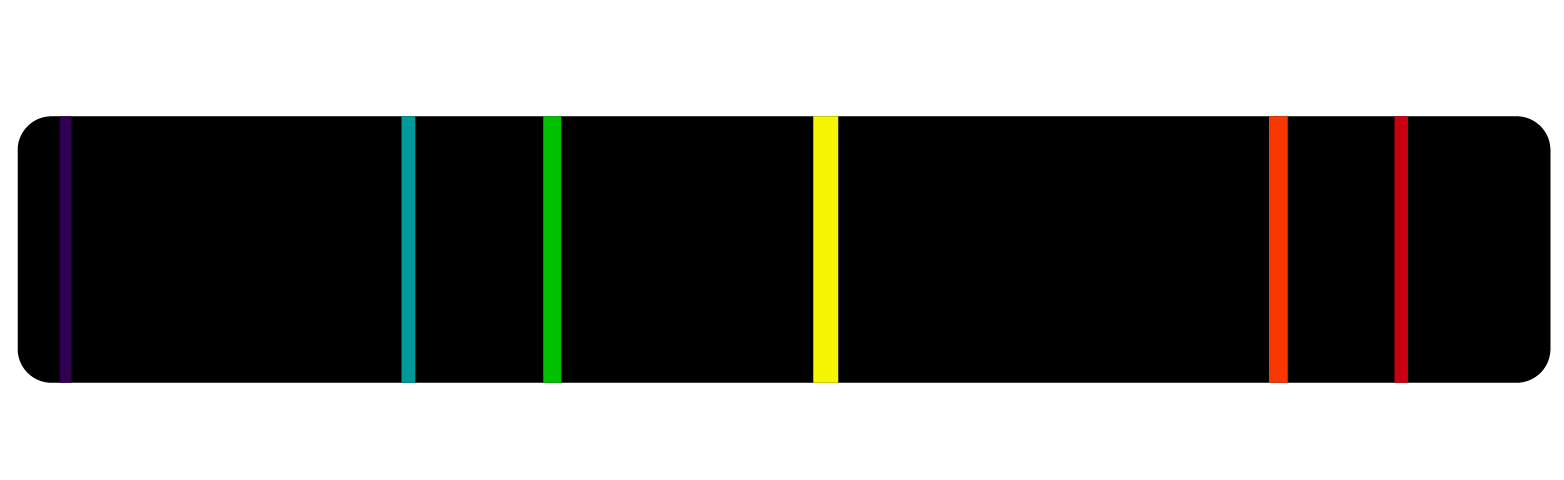
Emission spectrum of helium. When an electrical discharge is passed through a substance, its atoms and molecules absorb energy, which is reemitted as electromagnetic (EM) radiation, most commonly as visible light. The discrete nature of these emissions implies that the energy states of the atoms and molecules are quantized. Such atomic spectra were used as analytical tools for many decades before it was understood why they are quantized.
TUNNELLING
Imagine trying to roll a ball over a hill. Our experience tells us if we don't roll the ball with enough speed, it will not get over the hill.
Quantum physics tells us if the ball was a quantum particle, there would be no need for speed. Due to its wave-like behaviour, there is a small chance it could go through the hill and appear on the other side! We call this effect quantum tunnelling.

DECOHERENCE
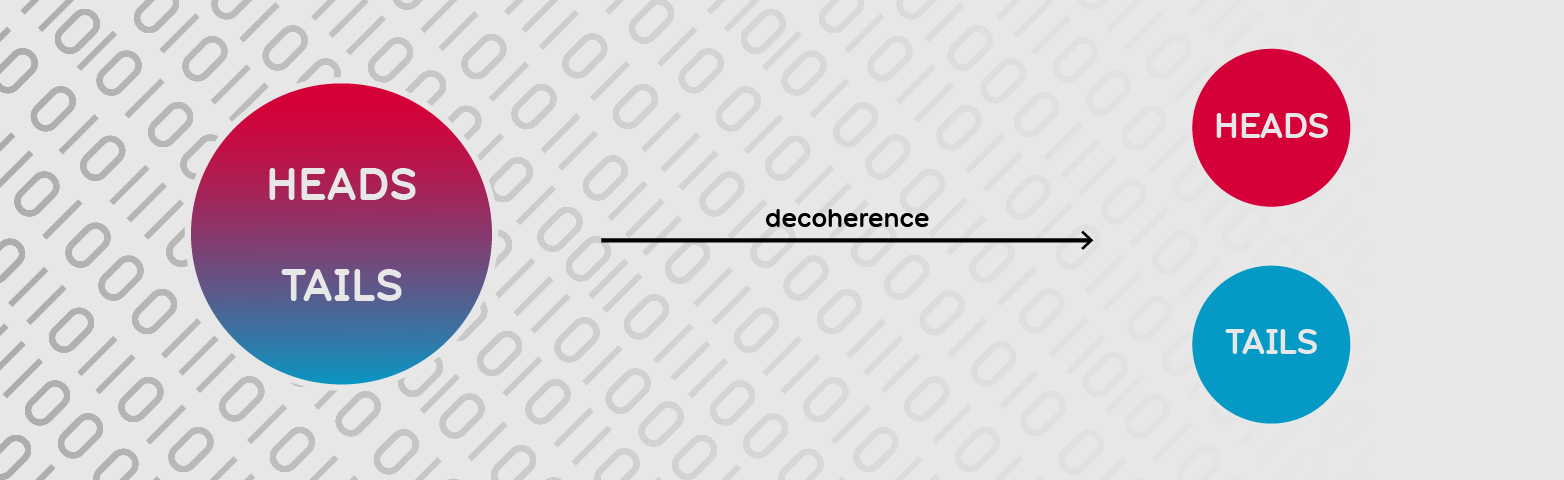
Quantum states are delicate. Unwanted interactions with their surroundings can affect a superposition, causing the state to collapse as if it’s being measured. We call this decoherence.
We can fight decoherence by isolating quantum particles from their surroundings through cooling or putting them in a vacuum.
Although decoherence is troublesome, we can still use it to our advantage to create ultra-sensitive sensors.
One way scientists battle decoherence is by cooling quantum particles to very low temperatures. At a fraction of a degree above the absolute zero, the environment is very still. This allows quantum particles to stay in superposition states for much longer, and allows us to control them more easily and with higher precision.
ENTANGLEMENT
When you can tell something about one object by looking at another, we call those objects correlated. For example, if you pull a left shoe out of a shoebox, you can be pretty sure the remaining shoe will go on your right foot.
Quantum particles can share a special sort of correlation called entanglement, where the two objects are so strongly correlated that the properties of one cannot be described without considering the properties of another. Instead of being described by two separate wavefunctions, entangled objects are described by a single joint wavefunction.
Entanglement describes a superposition state of multiple quantum particles, such as two electrons. While the properties of the electrons may be individually highly uncertain, their properties when measured together may be incredibly predictable. For example, two position-entangled electrons may have wavefunctions that spread over a large space, but when we measure one electron, we instantly know exactly where the other one is. Entanglement can be seen between quantum particles even if we separate them by vast distances, with some experiments showing entanglement surviving over hundreds of kilometres.
THE DOUBLE-SLIT EXPERIMENT
The double-slit experiment is a cornerstone example in quantum mechanics that neatly ties together concepts from superposition, interference, uncertainty, measurement and quantization.
When light passes through a narrow object, like a thin slit, it spreads out. When we place two thin slits side-by-side, the light passing through one spreads into the light from the other. By looking at the light far from the slits, we can see regions of constructive interference where light is more intense, and regions of destructive interference where there is no light at all. We can understand this as the light waves that spread from the left slit interfering with the light waves spreading from the right slit.
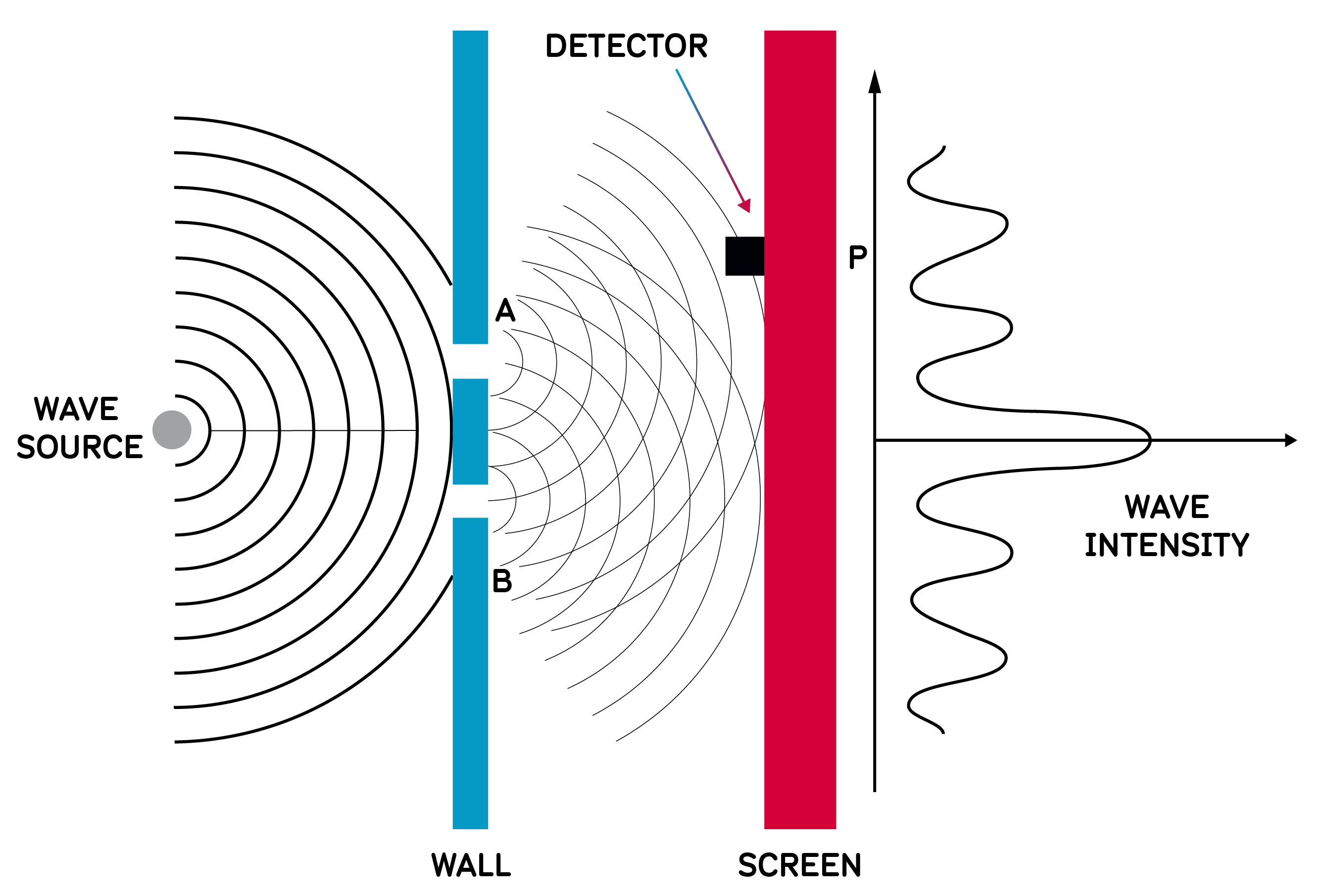
But from quantization, we know that light is emitted in discrete chunks called photons. What happens if we send a single photon into the double-slit experiment? If we measure which slit the photon goes through by placing a photon detector right after each slit, we see that it only goes through one slit or the other. But if we measure far away from the slits, we see an interference pattern that builds up slowly, one photon at a time:
Video Source: P. Kolenderski et al. (IQC/UWaterloo), Scientific Reports 2014; 4:4685. (Jennewein lab)
These are the two golden rules of quantum mechanics in action. The photon went through a superposition of both slits, and the wavefunction from the left slit interfered with the wavefunction from the right slit. Unlike the classical double-slit experiment, it is not the wave energies that interfere, but rather the wavefunction probabilities.
However, if we measure which slit the photon goes through, we see it in one slit or the other, never in both at the same time. Measuring which slit the photon goes through disturbs the superposition and destroys the interference. Indeed, precisely knowing which slit the photon went through and which interference pattern we see at the same time is impossible, an example of the uncertainty principle in action!